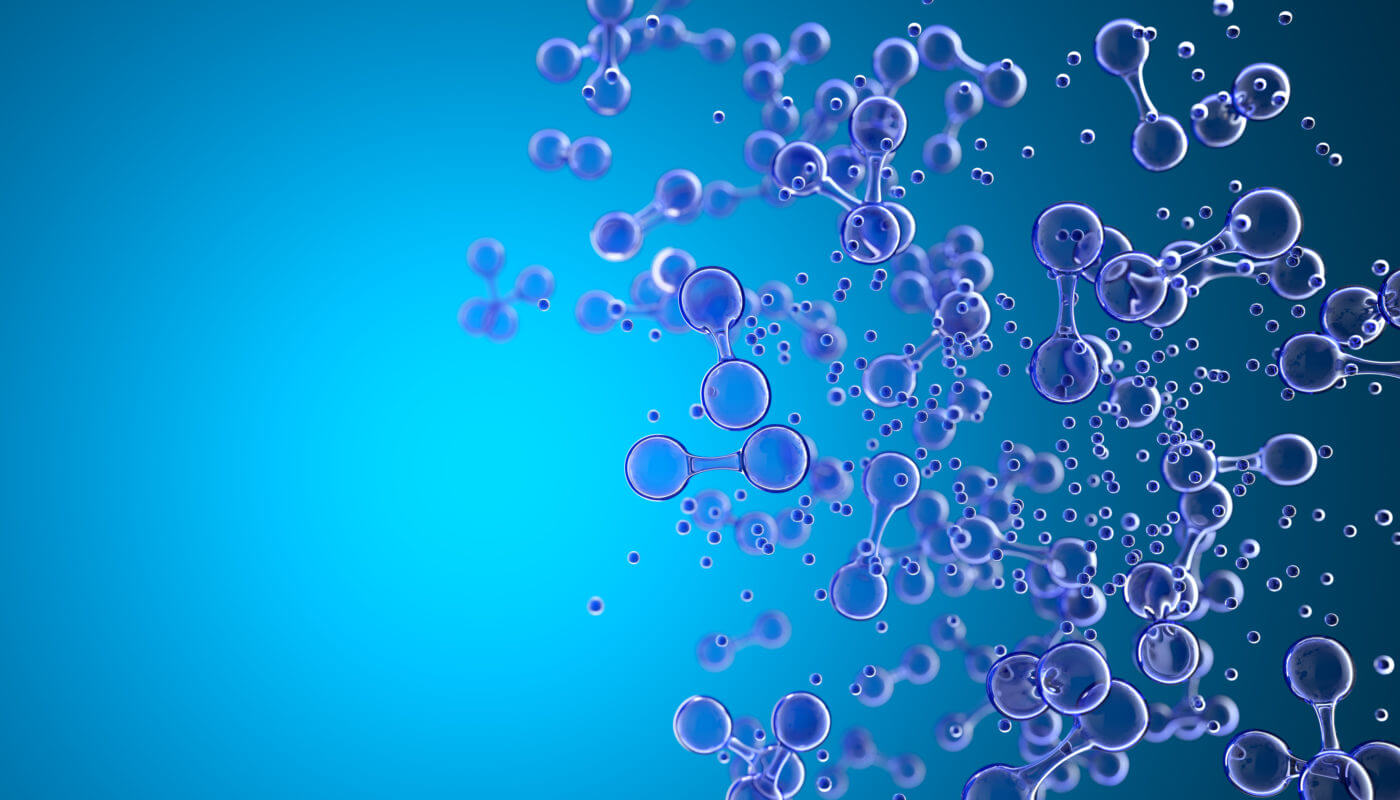
Assessing hydrogen emissions across the entire lifecycle
Currently, 80% of the world’s total final energy consumption is delivered through hydrocarbon molecules like coal, natural gas, and petroleum derivatives. Plans to decarbonize our energy system focus on electrifying most end-uses and providing that electricity with clean energy sources. However, some sectors will be difficult to electrify and zero-carbon fuels like hydrogen and ammonia can play a major role in replacing fossil fuels.
Global hydrogen demand is expected to increase from 90Mt/year to 530Mt/year by 2050, while in the EU, a seven-fold rise in hydrogen energy demand is expected by 2050 to help decarbonize hard-to-electrify sectors such as steelmaking, heavy transport, and energy-intensive industries. However, just like for all production processes, how hydrogen is generated has a large impact on its greenhouse gas (GHG) intensity; hydrogen must be produced by low-carbon energy sources in order to meet global climate goals and provide effective decarbonization of the energy system.
Hydrogen can be produced in several ways. Today, the vast majority of hydrogen is produced from fossil fuels, mainly from natural gas via methane reforming (commonly known as grey hydrogen). In the EU Gas Package, which is the European Commission’s proposal to decarbonize existing gas networks and regulate the nascent renewable and low-carbon hydrogen market, low-carbon hydrogen has been defined as hydrogen derived from non-renewable sources, meeting a greenhouse gas emission reduction threshold of 70% compared to a fossil fuel comparator. This definition of low-carbon hydrogen would cover, for instance, hydrogen produced via methane reforming with carbon capture and storage (SMR+CCS), commonly known as blue hydrogen, or hydrogen produced from nuclear energy. This does not include renewable hydrogen (usually called green hydrogen), which is produced using renewable energy sources such as PV and wind and is given a different category by the Commission.
Given the number of ways in which hydrogen can be produced, it is important to evaluate hydrogen production emissions through reliable and accurate GHG accounting considering emissions generated across the full lifecycle in order to compare the different production pathways effectively.
What is a Lifecycle Assessment and why is it important?
Lifecycle Assessment (LCA) is a method that quantifies the effects of a certain product in the environment by evaluating all the GHG emissions arising over its entire value chain and lifetime. Although zero-carbon fuels do not emit any CO2 when combusted, there are other important GHG emissions released across the fuel lifecycle that must be accounted for.
A recent study shows that at a low methane leakage rate of 0.2% and a high carbon capture rate for the methane reforming process can lower emissions by up to 75% compared to a system with a similar leakage rate but no CO2 capture. However, a higher methane leakage rate of 8% entirely overwhelms the benefit of CO2 capture: using a GWP100, overall emissions are similar to low leakage systems with no carbon capture and storage, and using a GWP20, emissions are nearly double systems with low leakage and no carbon capture and storage. This underscores the need to both implement carbon capture and storage and reduce upstream methane leakage.
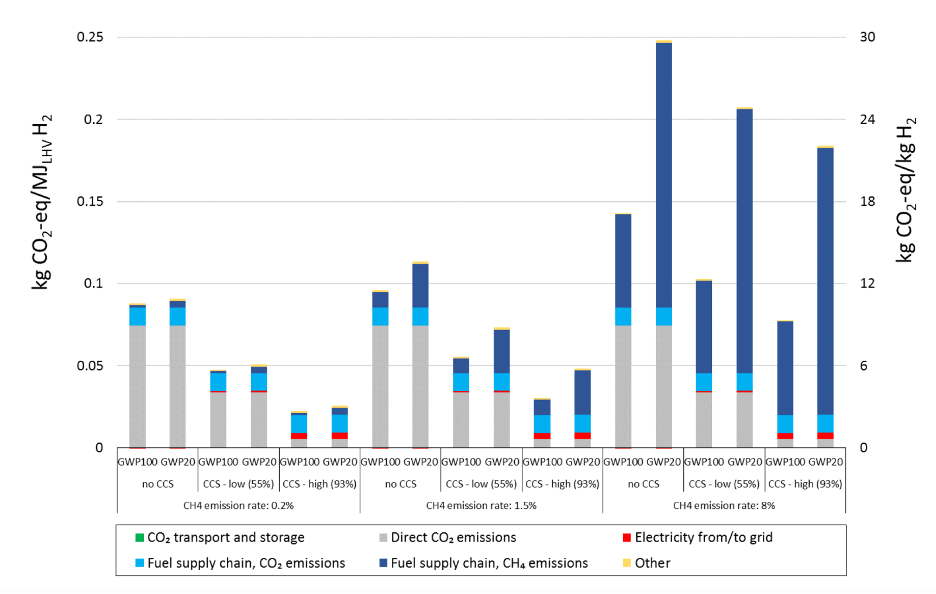
How is a LCA conducted?
The first step in performing a LCA is to establish the system boundaries, within which all GHG emissions will be accounted for. The figure below illustrates an example of a full LCA of different hydrogen production pathways (SMR+CCS and electrolysis). It covers all the stages from the production of primary energy (natural gas or electricity) to the final use of the fuel.
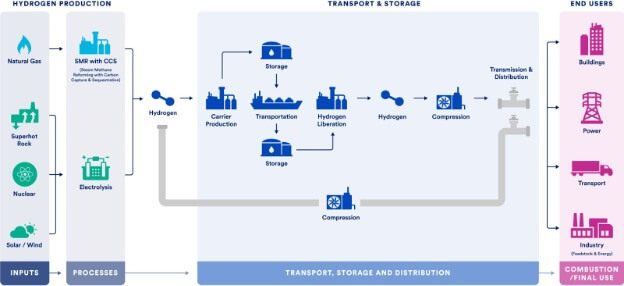
Stages to consider in an LCA
- Inputs refer to emissions from the production of all energy and materials needed for the fuel production process, including emissions from the construction of facilities. In the case of an SMR process, the energy input would be natural gas, while in the case of using an electrolyser for renewable hydrogen production, the input would be renewable electricity. In the case of natural gas, input emissions would include the emissions associated with natural gas extraction, processing, storage, and transport to the SMR plant, including any methane vents and leaks and CO2 emissions associated with flaring and combustion along the supply chain. Methane leakages can be an important contributor to the total GHG emissions of low-carbon hydrogen via SMR+CCS since the global warming potential of methane is 29.8 to 82.5 times higher than CO2 when accounted over a 100-year period (GWP100) or a 20-year period (GWP20). The GWP100 is commonly used in legislations for CO2 and methane, however, a shorter methane GWP such as the GWP20 would be more appropriate when evaluating these leakages to better reflect the short-term climate impact of methane, as the gas only stays in the atmosphere for about a dozen years but has a large impact on temperatures in the next decade.
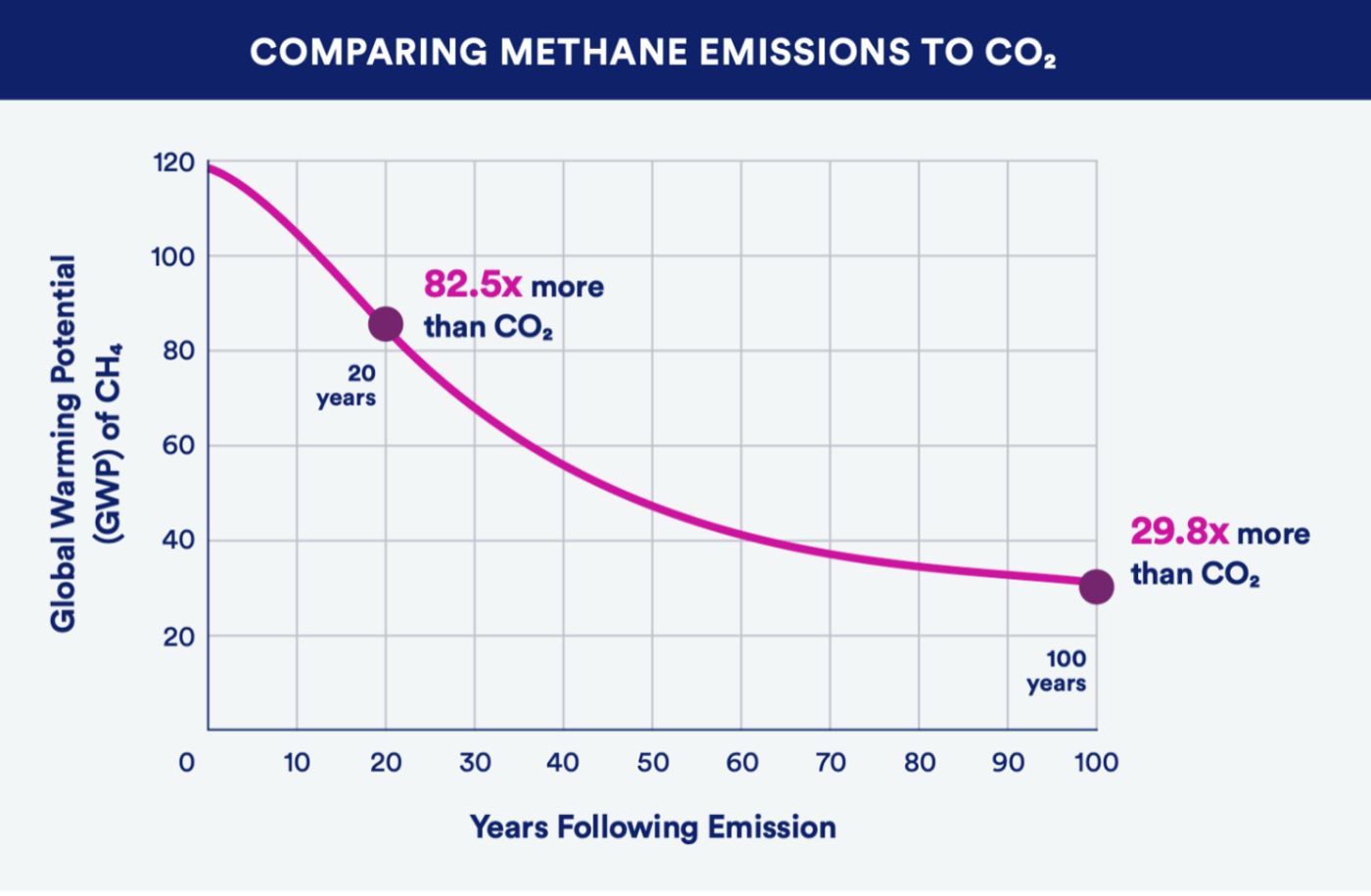
To produce low-carbon hydrogen via a natural gas pathway, carbon capture and storage is coupled with traditional steam reforming processes to capture the flue gases and reduce the total CO2 emissions. In this case, the extra energy used to run the carbon capture and storage unit (e.g.: electricity and heat) with its associated upstream emissions must also be accounted for as inputs. In the case of electrolytic hydrogen, emissions from the electricity production should also be considered, whether it is using electricity form the grid or renewable energies. Renewable energies are often referred as “zero-emissions electricity” since there are no combustion-associated emissions. However, upstream emissions from raw materials extraction and construction could be significant and shall be taken into account in the inputs as it is with any other energy source. The carbon footprint of renewable energies such as wind and PV depends strongly on capacity factors due to the strong variability of these resources, highly dependent on location. For instance, the carbon footprint of PV in Europe ranges between 38 gCO2e/kWh and 89 gCO2e/kWh, depending on the solar irradiation at the different locations. For wind energy, the median lifecycle GHG emission estimate for onshore and offshore is 11 g CO2eq/kWh.
- Processes refer to emissions associated with the process to generate the fuel (i.e.: the hydrogen production unit itself). In the case of hydrogen produced via SMR, these include emissions from the flue gases of the SMR unit. When combining SMR with carbon capture and storage, the emissions arising from the capture, transport, and storage processes that have not been included as part of the inputs (e.g.: CO2 leakages) must be also included; together with a negative emissions credit equal to the amount of CO2 captured and stored. For hydrogen produced using electrolysis, there are no emissions at this stage.
- Transport, storage, and distribution includes all the emissions generated between hydrogen production and the end user of the fuel. This includes emissions from construction of the required equipment and facilities as well as emissions from the energy used for transport, whether it is fuel for a maritime tanker or compression needed for distribution pipelines. Any upstream emissions associated with the production of the energy needed for transport need to be accounted for (e.g.: liquified natural gas used as the bunker fuel). If hydrogen is liquified for transport or a hydrogen carrier is used (e.g.: ammonia or liquid organic hydrogen carrier), all the emissions from the conversion/reconversion must also be included in this stage. A high-emissions transport and distribution system could potentially outweigh the benefits obtained from the use of zero-carbon fuels, even in the case of renewable hydrogen (e.g.: long-distance hydrogen transport through heavy fuel oil ships).
- Combustion/final use is the stage when the fuel is combusted to provide energy releasing its carbon content to the atmosphere. Zero-carbon fuels like hydrogen do not contain carbon atoms and therefore do not emit CO2 when combusted, as opposed to fossil fuels. However, during hydrogen or ammonia combustion, nitrogen oxides (Nox) can be formed as it happens when fossil fuels are combusted with air. Although Nox themselves are not greenhouse gases, they lead to the formation of ozone through secondary reactions causing an indirect GHG effect. The magnitude of this impact should be evaluated in the combustion emissions. In the case of Fuel Cell Electric Vehicles (FCEV), hydrogen is converted into electricity releasing only water.
Low-carbon vs. renewable hydrogen?
There is a long debate over low-carbon hydrogen, and in particular hydrogen from methane reforming processes with carbon capture and storage vs. renewable hydrogen due to the aforementioned methane leakages throughout the natural gas supply chain. Electrification and massive renewable energy deployment is at the core of the EU’s decarbonization strategy. However, renewable hydrogen alone will most likely not be able to cover all the hydrogen demand expected in the future, as has been recognized by the European Commission in the hydrogen strategy. Furthermore, when considering lifecycle emissions of renewable hydrogen, the associated upstream emissions of renewable energies shall also be considered, resulting in very low emissions hydrogen, but not zero emissions.
This is why a proper LCA of the different hydrogen production pathways is of utmost importance. In order for hydrogen through SMR+CCS to be considered truly ‘low-carbon,’ there are two key points that must be addressed:
- Minimizing methane emissions rates along the natural gas supply chain (ideally <0.3% for GWP20). In large gas producing countries that export gas to the EU—like Russia, Algeria, and the U.S.—typical emissions rates reach 2% or even 6%-8% in countries like Libya, Iraq, and some oil-heavy fields in the U.S. Lower methane loss rates between 0.003% and 1.3% were measured in Norwegian offshore O&G fields in 2019. CATF has documented feasible, and cost-effective methane standards that can be implemented to significantly reduce methane emissions in Europe and the U.S.
- Achieving high CO2 removal rates in methane reforming processes (>93%) Certain methane reforming processes like Authothermal Reforming (ATR) technologies allow a higher capture rate than SMR when coupled with carbon capture and storage. ATR technologies with high capture rates are is still under development but the “HyNet LCH” project in the UK is aiming for a total capture rate of 97% (with possibilities to increase this rate further) becoming operational by 2024.
As the world looks to establish a new global hydrogen market, European policymakers find themselves in a position to accelerate that process. With the massive projected need for imported hydrogen to decarbonize European industry and the major ports, and industry actors able to signal demand for low-carbon hydrogen production, Europe is a crucial marketplace for this new global commodity. On the policy side, establishing clear standards for hydrogen that ensure this new trade is climate beneficial would have massive decarbonization impacts even beyond Europe.
CATF is advocating for the adoption of LCA backed standards as soon as feasible and is working with legislators in Brussels and the EU capitals to ensure the coming hydrogen boom drives emissions down while safeguarding those sectors reliant on fuels today.